
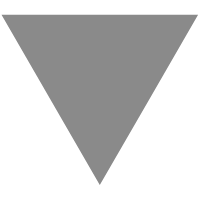
Double ridge formation over shallow water sills on Jupiter’s moon Europa
source link: https://www.nature.com/articles/s41467-022-29458-3
Go to the source link to view the article. You can view the picture content, updated content and better typesetting reading experience. If the link is broken, please click the button below to view the snapshot at that time.
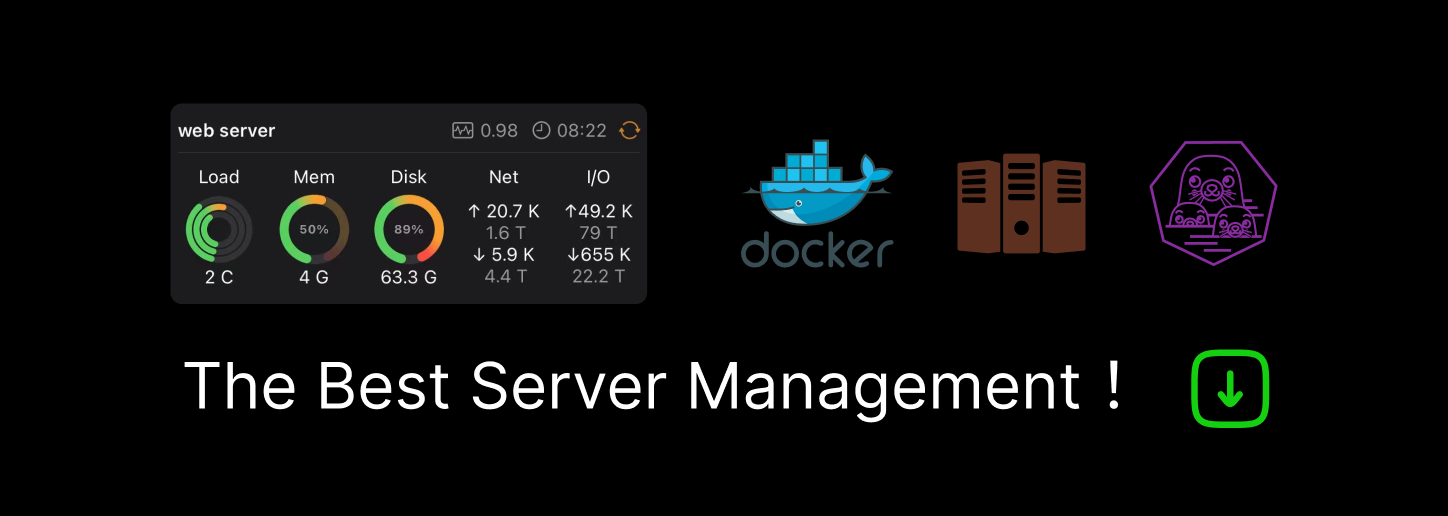
Introduction
Jupiter’s icy moon Europa harbors a global subsurface ocean beneath an outer ice shell1,2,3. The thickness and thermophysical structure of this ice shell are poorly constrained, but models suggest it may be 20–30 km thick4,5,6,7,8 with a layer of warm, convecting ice underlying a cold, rigid crust9,10. The detailed structure and dynamics of its ice shell and the timescales over which they evolve are critical for understanding both the fundamental geophysical processes and habitability of Europa11. Some of the primary observational constraints on these subsurface processes are their expressions in the surface morphologies imaged by Voyager and Galileo.
Europa’s surface is young12 and geologically active13,14, displaying a wide variety of landforms including ridges, troughs, bands, lenticulae, and chaos terrain15. Of these, double ridges are the most common, consisting of quasi-symmetric ridge pairs flanking a medial trough15,16, with height to peak-to-peak distance ratios <0.5817. These ridges may extend for hundreds of kilometers and include some of the oldest features visible on the surface, with frequent cross-cutting implying numerous formation cycles over Europa’s history15,16. Cycloidal ridges and ridge complexes share many of these characteristics and along-strike transitions between ridge morphologies are not uncommon, suggesting that a single process may be active in the formation of all ridge types18,19. Proposed formation mechanisms for double ridges fall into six categories: cryovolcanism20, tidal squeezing13, diapirism21, compression22, dike intrusion or ice wedging23,24, and shear heating25,26. All of these mechanisms require ice-shell fracture and, with the exception of compression and diapirism, all invoke near-surface ice-water interactions, either through internal melting26 or direct injection from the subsurface ocean13,20,24.
More recently, a number of extensions to the explosive cryovolcanism hypothesis have been proposed, in part to address the difficulty of driving negatively buoyant ocean water directly to the surface27. These models suggest that double ridges may instead form above shallow, crystallizing water bodies, such as sills or dikes, within the ice shell, rather than by direct connection to the subsurface ocean19,28,29,30. Such mechanisms are more consistent with morphometric analyses that both disfavor compressional formation models and support the presence of subsurface water reservoirs beneath ridges28. These models have much in common with formation mechanisms for lenticulae, chaos, and cratered terrain that invoke the emplacement and refreezing of shallow water bodies to explain the observed doming, surface disruption, or collapse31,32,33. This growing body of work suggests that shallow water may be critical, not only to double ridge formation, but also to Europan ice-shell dynamics, exchange, and ultimately habitability.
Analogs for double ridges or confined shallow water bodies from the terrestrial cryosphere could place powerful constraints on this hypothesis space for Europa34. Sea-ice pressure ridges35 and some ice rise divides36 bear a qualitative resemblance to Europa’s double ridges, but the pressure ridge analog assumes a very thin ice shell and ice divides express a flow regime entirely dissimilar to the Europan environment. Similarly, subglacial volcanic craters and ice shelf brine infiltration have been invoked as analogs for pressurized ice-water interactions32, but neither fully captures the physics of refreezing water bodies confined within an ice matrix.
Here, we present an icy double ridge discovered on the Greenland Ice Sheet with the same gravity-scaled geometry as Europa’s double ridges (Fig. 1). High-resolution ice penetrating radar observations reveal that this ridge is underlain by a shallow refreezing water sill (Fig. 2) and provide a direct window into the subsurface processes that drove its formation.
a Europan double ridge in a panchromatic image from the Galileo mission (image PIA00589). The ground sample distance is 20 m/pixel. b Greenland double ridge in an orthorectified panchromatic image from the WorldView-3 satellite taken in July 2018 (© 2018, Maxar). The ground sample distance is ~0.31 m/pixel. Signatures of flexure are visible along the ridge flanks, consistent with previous models for double ridges underlain by shallow sills28.
The color scale shows the calibrated received radar power with darker colors indicating a stronger return. The dashed blue box outlines the region shown in more detail in Fig. 3. This radargram shows the full crystallizing sill perched within the porous firn beneath the ice slab and resting on a base of impermeable meteoric ice. The water table is marked by a strong, horizontal, subsurface reflection below which no structure is visible due to the high attenuation. A clear split in the water table is visible directly beneath the double ridge. The surrounding region of low reflectivity is the refrozen ice shell.
Results
Greenland observations
This double ridge is located ~60 km inland of the ice-sheet margin in Northwest Greenland (Supplementary Fig. 1) at 74.566° N, 54.0531° W. ArcticDEM digital elevation models (DEMs) (Supplementary Fig. 2) and ICESat-2 satellite laser altimetry measurements (Fig. 3d) show that an asymmetric precursor ridge system developed by July 2013, with the full double ridge forming by March 2016 and persisting to the present day. The most recent DEM, collected in March 2016, shows two ~800 m long quasi-symmetric ridges flanking a central trough. The average ridge height is 2.1 m, with the northern ridge ~1.4 times the height of the southern ridge. The peak-to-peak distance varies from 26 to 78 m along-strike, with an average width of 46 m. After scaling the mean ridge height by the ratio of Earth’s gravity to Europa’s, the average height to peak-to-peak distance ratio is 0.37, entirely consistent with Europan ridge geometry17. Surface imagery and the DEMs also show evidence of flexure along the ridge flanks (Fig. 1, Supplementary Fig. 2), similar to ridges observed on Europa28.
In panels a–c, the color scale shows the relative received radar power with darker colors indicating stronger returns. In panel d, the surface elevation profiles are color-coded by collection date. a Radargram collected in May 2015. The water table is continuous and the southern ridge has not yet developed. b Radargram collected in May 2016. This radargram shows the full double ridge, underlain by the divided and upwelling water table, along with scattering from likely void space beneath the central trough. c Radargram collected in March 2017. The system morphology remains generally stable, with reduced reflectivity in the upwelling water table and void spacing, suggesting freeze-out and creep closure. d ICESat-2 surface elevation profiles collected over the ridge in October 2018 (dark purple), April 2019 (light purple), and January 2020 (orange). These plots show high-quality (level 4) photons from ground track 331. Apparent changes in ridge cross-section may be the result of along-strike variations in ridge structure as the ridge is advected ~106 ma−1 under the fixed satellite track.
The double ridge is located within the percolation zone where the ice-sheet near-surface consists of a layer of porous, compacting snow known as firn that reaches the density of solid ice some tens of meters below the surface. During the summer melt season, surface meltwater percolates into this porous layer, where it can refreeze to form multi-meter-thick, horizontally continuous ice slabs, extensive ice layers (0.1–1 m thick), or smaller ice lenses (<0.1 m thick) with limited horizontal extent. Previous work has shown that the subsurface structure in this area typically consists of a 3–5 m thick low-permeability ice slab perched above a partially porous firn layer with significant ice lensing that transitions to solid ice at a depth of 30–40 m37,38. At the local scale, the ridge is situated in the bottom of closed surface basin with an average ice velocity of 106 ma−1, flowing approximately parallel to the strike of the ridge.
Ultrawideband airborne radar sounding data collected in May 2016 as part of NASA’s Operation IceBridge using the Center for Remote Sensing of Ice Sheets (CReSIS) Multichannel Coherent Radar Depth Sounder (MCoRDS) reveal that this double ridge is underlain by a shallow water sill (Fig. 2). This cross-sectional subsurface image, oriented transverse to the ridge strike, displays anomalously bright subsurface echoes consistent with reflections from a water table located 10–15 m below the ice surface and extending laterally for ~1.6 km (Methods). This sill is well below the typical thickness of the overlying refrozen ice slabs37, suggesting that it was originally emplaced within the relict porous firn layer. However, a localized surface uplift of up to 9 m associated with sill emplacement (Supplementary Fig. 3), as well as strong hyperbolic scattering from the water table (Supplementary Fig. 4), suggests that this water may occupy a macro-porous fracture or conduit network rather than simply saturating pre-existing pore space. Based on the displaced surface volume, we estimate the initial water volume was at least 7.2 × 106 ± 1.7 × 106 m3 (Methods). The sill is surrounded by an ellipsoidal region of extremely low relative radar reflectivity and minimal stratigraphic structure, consistent with a homogeneous region with minimal internal contrast in material density (Fig. 2). This suggests that the sill is encased within a refrozen ice mass that extends to a total width of ~2.4 km and a depth of about 25 m below the surface. Pore close-off is estimated to occur at ~30 m depth in this region38, so this sill likely rests on an impermeable base of meteoric ice. We also observe a distinct upwelling of the water table directly beneath each of the ridges, with particularly low radar reflectivity beneath the central trough, suggesting a break in the sill.
To constrain the degree to which each region has been modified by surface meltwater infiltration and refreezing, we use a Markov Chain Monte Carlo inversion with a radar scattering forward model to map the distributions of radar reflectivity within the firn, ice shell, and sub-ridge regions to estimates of the fractional refrozen ice volume in each area (Supplementary Fig. 5, Methods). This also provides a proxy for porosity, with higher ice content reflecting lower porosity. Consistent with our interpretation that the sill is encased in a refrozen ice shell, we find an ice fraction of 0.8 (0.27) (median and interquartile range) for the low reflectivity regions surrounding the sill, compared to 0.65 (0.28) in regions identified as firn. We interpret an additional 5 dB reduction in radar reflectivity directly beneath the ridge system, corresponding to a median ice fraction of 0.86 (0.23), to show that the sill was split by refreezing within a vertical conduit or fracture network, concentrating subsequent water injection from the sill into the firn directly to either side of this now impermeable barrier.
We directly observe these subsurface processes associated with ridge formation in a series of high-resolution radargrams collected by the CReSIS Snow Radar between May 2015 and March 2017 (Fig. 3). In the May 2015 data (Fig. 3a), we observe a highly asymmetric system with a prominent northern ridge ~2 m in height and minimal evidence for a southern ridge. The water table is relatively continuous, peaking to the north of the ridge, and the sub-ridge upwellings have not yet developed. The May 2016 data (Fig. 3b) shows a clear double ridge with the divided sill and symmetric upwellings directly beneath each ridge. This radargram also shows a new set of stacked point scatterers beneath the central trough and capping the divide in the sill (Supplementary Fig. 4). These signatures are consistent with scattering from some form of englacial void space (Methods), supporting our interpretation that the sill was divided after water refroze within a central vertical conduit or fracture network. The March 2017 data (Fig. 3d) reflects a similar morphology, although we observe small variations in the ridge height and a reduction in the relative reflectivity of the upwelling portion of the water table as well as the central point scatterers that could be consistent with ongoing refreezing or creep closure of voids. However, given that the ridge is advected past the fixed radar flight path at a rate of ~106 ma−1 and the spatial variations in ridge height and width observed in the 2016 surface DEM, these changes in observed morphology and reflectivity may reflect along-strike variability in ridge structure, rather than a true change in ridge structure with time.
Ridge formation mechanism
These observations reveal a refreezing-driven mechanism behind the formation of this double ridge (Fig. 4). In the first stage, a shallow water sill is emplaced within a porous matrix perched on a much thicker base of impermeable ice (Fig. 4a). In Greenland, seasonal melting, surface topography, and stress regime likely played critical roles in sill emplacement. We infer that during the 2012 extreme melt season, meltwater flowed laterally over the ice slab surface into the surface basin hydraulic low before ultimately draining into the subsurface through fractures in the overlying ice slab to form the sill observed in the radar data (Methods). Leak-off into the porous firn layer may have reduced the water pressure within the fracture enough to prevent fracture propagation to the ice-sheet bed as is common in similar closed basins in Greenland’s ablation zone. However, mechanical failure of the overlying firn or ice slab during injection and uplift may have led to the formation of the initial fracture observed near the edge of the uplifted region (Supplementary Fig. 2b).
Gray areas are ice, white/light gray shows pore space, and blue areas are liquid water. Red arrows show the direction of forces acting on the sill and the direction of water flow if relevant. a Stage 1—sill emplacement. In Greenland, this likely occurs through concentrated drainage of surface meltwater41. On Europa, sills might form by direct injection from the subsurface ocean27,29,43, from melting over rising diapirs32,44, or through shear heating26,30. b Stage 2—the sill fractures due to internal overpressure or other near-surface stresses and water fills the central conduit. c Stage 3—the sill is divided by refreezing within the central conduit or fracture network. d Stage 4—a double ridge develops as overpressurized water within the refreezing sill is forced out along the planes of weakness flanking the refrozen central conduit, producing the symmetric surface doming.
In the second stage, inflow or melting ceases and the sill begins to refreeze from its outer boundaries inward, eventually leading to upward-propagating fractures in response to overpressure in the confined water body31,39 (Fig. 4b). In Greenland, overpressure may have reactivated the initial fracture observed in 2013. Water then rises within these fractures due to the pressure gradient and, in stage three, refreezes, forming an impermeable ice plug that divides the sill in two (Fig. 4c). In Greenland, although this initial fracture formed along the edge of the sill, the system still equilibrated to the classic quasi-symmetric double ridge morphology, except where the fracture was complex or discontinuous. This suggests that ridge symmetry may be a result of the internal physics of formation rather than the initial geometry of the system, and that this mechanism is robust to fracture position and orientation so long as some minimum water volume is retained within both fragments of the divided sill. Similarly, if some hydrologic connection is maintained between the two sill segments, either because the central refrozen conduit does not extend the full depth of the sill or because it is discontinuous along-strike, this would ensure that the pressure gradient is similar on both sides of the central refrozen conduit, leading to similar degrees of surface extrusion. While we also observe a transition from single ridge to double ridge in Greenland during these stages, it is not clear if this evolution is an innate feature of the mechanism we describe here, or specific to some local condition such as an initial asymmetry in pressure between the two sill segments or the external influence of transverse compression in this surface basin. The single ridge is unlikely to be an expression of the central refrozen conduit itself, given that there is no clear mechanism for such a ridge to then subside to form the medial trough between the double ridges. However, our observations do suggest that it is possible for single ridges to later evolve into double ridges under these general conditions.
In the final stage, ongoing refreezing continues to raise the pressure in the sill, producing secondary fractures along the existing planes of weakness at the rheologic boundaries to either side of the refrozen central conduit (Fig. 4d). This creates high permeability pathways into the overlying porous matrix, and water preferentially flows along these paths in response to the pressure gradient, creating the observed upwellings and injecting water into the overlying material. A combination of volume expansion as this water refreezes, the subsurface pressure gradients within the sill itself, and lateral compression from refreezing in the central conduit19 ultimately force the softer, porous material up and out along the weak points to either side of the refrozen central conduit, extruding at the surface to form the double ridge. In this model, volume expansion from refreezing likely generates the added volume of the ridges. In Greenland, approximately \(18.6 \% \genfrac{}{}{0ex}{}{+5.7 \% }{-3.5 \% }\) of the initial sill volume would need to refreeze to form the ridges as observed in 2016, consistent with the large refrozen ice shell we observe around the liquid water table. Widespread surface subsidence over the sill or sill recharge during the melt season could also allow for some of the implied redistribution of mass or addition of volume. However, the registration accuracy of the available DEMs and poorly constrained accumulation, ablation, and ice flow processes preclude any reliable partitioning of surface elevation changes, which would be needed to assess whether these processes occurred in Greenland.
Greenland as a Europa analog
Our results suggest that the refreezing of confined aquifers in the near-surface of the Greenland Ice Sheet is a promising analog for the progressive pressurization and fracture of crystallizing shallow sills in the brittle lid of Europa’s ice shell. In particular, Greenland provides examples of the evolution of isolated liquid water pockets confined within a semi-permeable, subfreezing ice matrix and perched on an impermeable ice body of much greater thickness, similar to hypothesized conditions at Europa. However, significant differences in gravity, atmospheric pressure, temperature, and ice impurity content will likely lead to complex scaling relationships between the two environments. While the dearth of observations at Europa limits our capacity to constrain many of these variables, in the context of double ridge formation, we can assess the general viability of the physical processes we invoke and the qualitative impact of these environmental differences.
In general, the low gravity (1.3 m/s) and atmospheric pressure (0.1 μPa) at Europa should aid ridge formation. Both conditions act to reduce overburden on the sill, relaxing the overpressure conditions needed to drive water towards the surface or uplift the material overlying the sill. A smaller fraction of the sill might be required to refreeze to form the ridges in this case, but only if the degree of refreezing needed to achieve the minimum overpressure exceeded the degree of refreezing needed to generate the added ridge volume by expansion.
Ice temperature and chemistry are poorly constrained confounding factors at Europa that play an important role in governing ice rheology. Our model invokes some degree of near-surface porosity, suggesting that sills would need to form within or at the lower boundary of the porous portion of Europa’s ice shell. Previous work estimates this near-surface layer to be on average 3 km thick8,40, and most models of sill emplacement on Europa have assumed depths 1–5 km for sill intrusions19,28,29,31. This would place sills firmly within the brittle, conducting portion of the ice shell where temperatures likely do not exceed 150 K (ref. 8), except where latent heating can warm the surrounding ice. Therefore, we expect elastic deformation or brittle fracture to dominate in response to imposed stress, with little to no contribution from viscous creep. This is consistent with our proposed mechanism, which invokes fracture and rapid uplift or extrusion of damaged material to form the ridges, despite the significantly warmer conditions in Greenland (~260 K). However, the length scales and timescales for ridge formation would be strongly modulated by the details of the rheology.
These temperature differences also imply an absence of surface melting on Europa, whereas melt is common at the double ridge site in Greenland. In particular, Landsat 7 ETM+ and Landsat 8 OLI optical satellite imagery show visible surface runoff and localized water ponding within the basin during four out of the five summers spanned by our DEM and radar observations (Supplementary Fig. 6). Therefore, we consider two key ways in which the frequent presence of surface water might limit the value of the Greenland double ridge system as a Europa analog.
First, seasonal runoff might recharge the sill, either by activation of old fractures or formation of new fractures during the melt season. Sill replenishment would lower the total amount of refreezing required to form the new ridge volume and lengthen the refreezing time relative to a closed system, given the seasonal influx of additional mass and heat. However, this would not necessarily prevent the cycle of refreezing, fracture, and pressurization proposed for ridge formation, and in fact, sill replenishment from the subsurface ocean has also been considered as a viable mechanism for prolonging sill lifetime on Europa29.
Second, surface melt, stream incision, or preferential ablation beneath ponded water might actively rework Greenland’s surface topography, leading to ridge morphologies that would not be possible on Europa. However, we assess that it would be difficult to form isolated ridges by preferential downcutting of the surrounding terrain unless the ridges were already of a sufficient height to prevent ponding or flow overtop of them. Additionally, within a closed basin, heterogeneous melting should primarily redistribute topography laterally, making widespread surface lowering by downcutting difficult to achieve. We also observe a largely stable ridge morphology in the DEM, radar, laser altimeter, and surface imagery observations between 2016 and 2020, despite significant differences in annual accumulation, melt, and surface runoff from year to year (Supplementary Fig. 6). This suggests that the double ridges are unlikely to be predominantly shaped by ongoing seasonal melt or water flow. In general, it is more likely that surface processes would reduce, rather than heighten, the symmetry and along-strike consistency of the Greenland ridges, reducing rather an increasing the similarities with Europa’s ridges. The absence of these processes on Europa might explain the more consistent dimensions of the moon’s ridges.
Thus, despite the differences in environment, we suggest that Greenland can offer promising analogs for shallow water dynamics in the brittle lid of Europa’s ice shell. The Northwest Greenland marginal accumulation zone is of particular interest due to its abundance of near-surface refreezing and liquid water features, including ice slabs37, firn aquifers41, and buried supraglacial lakes42. This environment is a natural laboratory for studying the surface expressions and underlying dynamics of near-surface water reservoirs confined within a shallow, subfreezing porous ice matrix that transitions to impermeable ice with depth. Further investigations into the effects of water migration, refreezing, and fracture on the permeability and rheology of the subsurface, as well as the interplay between surface stresses and subsurface pressure gradients, may offer new insights into the dynamics of other Europan features such as lenticulae and chaos terrain.
Recommend
-
44
README.md ridge_map Ridge plots of ridges A library for making ridge plots of... ridges. Choose a location, get an elevation map, and...
-
6
October 14, 2021
-
9
Europa Clipper: What We Know About The Spacecraft That Will Travel To Jupiter's Moon In 2024 ...
-
9
Next Up How Particle Accelerators Hit The Big Time ...
-
12
NASA’s Juno Will Perform Close Flyby of Jupiter’s Icy Moon Europa As the spacecraft makes a close approach of the moon, it is expected to provide valuable science – and...
-
5
NASA shares first close-up images of Jupiter moon Europa in two decades Juno flew within 219 miles of Jupiter's moon By
-
3
NASA Study Suggests Shallow Lakes in Europa’s Icy Crust Could Erupt New research makes hypotheses that NASA’s Europa Clipper can test: An...
-
12
Painting the Water Tower
-
8
Appreciation for Running Water We were visiting relatives in Colorado when Dave's phone rang. Someone from county utilities, letti...
-
10
Carrying Water for a Canine Companion
About Joyk
Aggregate valuable and interesting links.
Joyk means Joy of geeK