
2
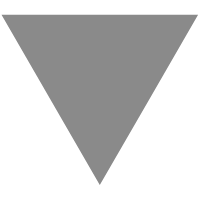
Measurement of the W boson mass reveals 7σ deviation from calculations
source link: https://www.science.org/doi/10.1126/science.abk1781
Go to the source link to view the article. You can view the picture content, updated content and better typesetting reading experience. If the link is broken, please click the button below to view the snapshot at that time.
High-precision measurement of the W boson mass with the CDF II detector
Expand for moreOPEN IN VIEWER
Expand for moreOPEN IN VIEWER
OPEN IN VIEWER
OPEN IN VIEWEROPEN IN VIEWER
OPEN ACCESS
Research Article
PARTICLE PHYSICS
Share on
High-precision measurement of the W boson mass with the CDF II detector
Science • 7 Apr 2022 • Vol 376, Issue 6589 • pp. 170-176 • DOI: 10.1126/science.abk1781
1
Metrics
Total citations1
Related Perspective
Weighing the W boson
W bosons mediate the weak interaction, one of the fundamental forces in physics. Because the Standard Model (SM) of particle physics places tight constraints on the mass of the W boson, measuring the mass puts the SM to the test. The Collider Detector at Fermilab (CDF) Collaboration now reports a precise measurement of the W boson mass extracted from data taken at the Tevatron particle accelerator (see the Perspective by Campagnari and Mulders). Surprisingly, the researchers found that the mass of the boson was significantly higher than the SM predicts, with a discrepancy of 7 standard deviations. —JS
Abstract
The mass of the W boson, a mediator of the weak force between elementary particles, is tightly constrained by the symmetries of the standard model of particle physics. The Higgs boson was the last missing component of the model. After observation of the Higgs boson, a measurement of the W boson mass provides a stringent test of the model. We measure the W boson mass, MW, using data corresponding to 8.8 inverse femtobarns of integrated luminosity collected in proton-antiproton collisions at a 1.96 tera–electron volt center-of-mass energy with the CDF II detector at the Fermilab Tevatron collider. A sample of approximately 4 million W boson candidates is used to obtain MW=80,433.5±6.4stat±6.9syst=80,433.5±9.4 MeV/c2, the precision of which exceeds that of all previous measurements combined (stat, statistical uncertainty; syst, systematic uncertainty; MeV, mega–electron volts; c, speed of light in a vacuum). This measurement is in significant tension with the standard model expectation.
The observation of the Higgs boson (1–4) at the Large Hadron Collider (LHC) (5, 6) has validated the last missing piece of the standard model (SM) (7–9) of elementary particle physics. This model, which incorporates quantum mechanics, special relativity, gauge symmetry, and group theory, currently describes most particle physics measurements with high accuracy. It postulates a number of experimentally established symmetries among particle properties, which tightly constrain the parameters of the model from experimental data (10). Given the current experimental precision and the predictive power of the SM, global fits of the model to the data render precise estimates of fundamental parameters, such as the mass of the W boson. As one of the mediators of the weak nuclear force, this particle is a key component of the SM framework. Its mass, one of the most important parameters in particle physics, is presently constrained by SM global fits to a relative precision of 0.01%, providing a strong motivation to test the SM by measuring the W boson mass to the same level of precision.
All fundamental particle masses, including that of the W boson, are generated in the SM through interactions with the condensate of the Higgs field in the vacuum. The formation of the condensate and the quantum excitation of this field, the Higgs boson (2–4), are parametrized but not explained by the SM. A number of hypotheses have been promulgated to provide a deeper explanation of the Higgs field, its potential, and the Higgs boson. These include supersymmetry—a spacetime symmetry relating fermions and bosons [(11) and references therein]—and compositeness, in which additional strong confining interactions produce the Higgs boson as a bound state [(12) and references therein]. Many of these hypotheses include a source of dark matter, which is currently believed to comprise ~84% of the matter in the universe (10) but cannot be accounted for in the SM. Evidence for dark matter is provided by the abnormally high speeds of revolution of stars at large radii in galaxies, the velocities of galaxies in galaxy clusters, x-ray emissions sensing the temperature of hot gas in galaxy clusters, and the weak gravitational lensing of background galaxies by clusters [(13, 14) and references therein]. The additional symmetries and fields in these extensions to the SM would modify (15–24) the estimated mass of the W boson (Fig. 1) relative to the SM expectation (10) of MW=80,357±4inputs±4theory MeV (25). The SM expectation is derived from a combination of analytical relations from perturbative expansions on the basis of the internal symmetries of the theory and a set of high-precision measurements of observables, including the Z and Higgs boson masses, the top-quark mass, the electromagnetic (EM) coupling, and the muon lifetime, which are used as inputs to the analytical relations. The uncertainties in the SM expectation arise from uncertainties in the data-constrained input parameters (10) and from missing higher-order terms in the perturbative SM calculation (26, 27). An example of a nonsupersymmetric SM extension is a modified Higgs sector that includes an additional scalar field with no SM gauge interactions, which predicts an MW shift of up to ~100 MeV (17), depending on the mass of the additional scalar particle and its interaction with the SM Higgs boson. A light (heavy) additional scalar particle would induce a positive (negative) MW shift. Similar but smaller shifts of 20 to 40 MeV have been calculated in an extension that contains a second Higgs-like field with the same gauge charges as the SM Higgs field (18). Implications of very weakly interacting new particles such as “dark photons” (19), restoration of parity conservation in the weak interaction (20), the possible composite nature of the Higgs boson (21), and model-independent modifications of the Higgs boson’s interactions (22–24) have also been evaluated.

Fig. 1. Experimental measurements and theoretical predictions for the W boson mass.
The red continuous ellipse shows the MW measurement reported in this paper and the global combination of top-quark mass measurements, mt=172.89±0.59 GeV (10). The correlation between the MW and mt measurements is negligible. The gray dashed ellipse, updated (16) from (15), shows the 68% confidence level (CL) region allowed by the previous LEP-Tevatron combination MW=80,385±15 MeV (45) and mt (10). That combination includes the MW measurement published by CDF in 2012 (41, 43), which this paper both updates (increasing MW by 13.5 MeV) and subsumes. As an illustration, the green shaded region (15) shows the predicted mass of the W boson as a function of the top-quark mass mt in the minimal supersymmetric extension (one of many possible extensions) of the standard model (SM), for a range of supersymmetry model parameters as described in (15). The thick purple line at the lower edge of the green region corresponds to the SM prediction with the Higgs boson mass measured at the LHC (10) used as input. The arrow indicates the variation of the predicted W boson mass as the mass scale of supersymmetric particles is lowered. The supersymmetry model parameter scan is for illustrative purposes and does not incorporate all exclusions from direct searches at the LHC. unc., uncertainty.
Previous analyses (28–44) yield a value of MW=80,385±15 MeV (45) from the combination of Large Electron-Positron (LEP) collider and Fermilab Tevatron collider measurements. The ATLAS Collaboration has recently reported a measurement, MW=80,370±19 MeV (46, 47), that is comparable in precision to the Tevatron results. The LEP, Tevatron, and ATLAS measurements have not yet been combined, pending evaluation of uncertainty correlations.
CDF experiment at Tevatron
The Fermilab Tevatron produced high yields of W bosons from 2002 to 2011 through quark-antiquark annihilation in collisions of protons (p) and antiprotons (ˉp) at a center-of-mass energy of 1.96 TeV. The (anti)quark momentum distributions in the (anti)proton are the best-measured among all constituent partons of the colliding particles. The use of proton-antiproton collisions reduces uncertainties on the momenta of the partons and the corresponding MW uncertainty relative to the LHC, where W bosons are produced from quarks or antiquarks and gluons, the latter of which have less precisely known momentum distributions. The moderate collision energy at the Tevatron further restricts the parton momenta to a range in which their distributions are known more precisely, compared with the relevant range at the LHC. The LHC detectors partially compensate with larger lepton rapidity coverage. The improved lepton resolution at the LHC detectors has a minor impact on the MW uncertainty. Although the LHC dataset is much larger, the lower instantaneous luminosity at the Tevatron and in dedicated low-luminosity LHC runs helps to improve the resolution on certain kinematic quantities, compared with the typical LHC runs.
The data sample corresponds to an integrated luminosity of 8.8 inverse femtobarns (fb−1) of pˉp collisions collected by the CDF II detector (43) between 2002 and 2011 and supersedes the earlier result obtained from a quarter of these data (41, 43). In this cylindrical detector [figure 3 of (43)], trajectories of charged particles (tracks) produced in the collisions are measured by means of a wire drift chamber (a central outer tracking drift chamber, or COT) (48) immersed in a 1.4-T axial magnetic field. Energy and position measurements of particles are also provided by EM and hadronic calorimeters surrounding the COT. The calorimeter elements have a projective tower geometry, with each tower pointing back to the average beam collision point at the center of the detector. Additional drift chambers (49) surrounding the calorimeters identify muon candidates as penetrating particles. The momentum perpendicular to the beam axis (cylindrical z axis) is denoted as pT (if measured in the COT) or ET (if measured in the calorimeters). The measurement uses high-purity samples of electron and muon (together referred to as lepton) decays of the W± bosons, W→eν and W→μν, respectively (e, electron; ν, neutrino; μ, muon).
W and Z boson event selection
Events with a candidate muon with pT > 18 GeV or electron with ET > 18 GeV (50) are selected online by the trigger system for offline analysis. The following offline criteria select fairly pure samples of W→μν and W→eν decays. Muon candidates must have pT > 30 GeV, with requirements on COT-track quality, calorimeter-energy deposition, and muon-chamber signals. Cosmic-ray muons are rejected with a targeted tracking algorithm (51). Electron candidates must have a COT track with pT > 18 GeV and an EM calorimeter-energy deposition with ET > 30 GeV and must meet requirements for COT track quality, matching of position and energy measured in the COT and in the calorimeter (ET/pT < 1.6), and spatial distributions of energy depositions in the calorimeters (43). Leptons are required to be central in pseudorapidity (|η|<1) (50) and within the fiducial region where the relevant detector systems have high efficiency and uniform response. When selecting the W boson candidate sample, we suppress the Z boson background by rejecting events with a second lepton of the same flavor. Events that contain two oppositely charged leptons of the same flavor with invariant mass in the range of 66 to 116 GeV and with dilepton pT < 30 GeV provide Z boson control samples (Z→ee and Z→μμ) to measure the detector response, resolution, and efficiency, as well as the boson pT distributions. Details of the event selection criteria are described in (43).
The W boson mass is inferred from the kinematic distributions of the decay leptons (ℓ). Because the neutrino from the W boson decay is not directly detectable, its transverse momentum pνT is deduced by imposing transverse momentum conservation. Longitudinal momentum balance cannot be imposed because most of the beam momenta are carried away by collision products that remain close to the beam axis, outside the instrumented regions of the detector. By design of the detector, such products have small transverse momentum. The transverse momentum vector sum of all detectable collision products accompanying the W or Z boson is defined as the hadronic recoil →u=ΣiEisin(θi)ˆni, where the sum is performed over calorimeter towers (52) with energy Ei, polar angle θi, and transverse directions specified by unit vectors ˆni. Calorimeter towers containing energy deposition from the charged lepton(s) are excluded from this sum. The transverse momentum vector of the neutrino →p νT is inferred as →p νT≡−→p ℓT−→u from →pT conservation, where →p ℓT is the vector pT (ET) of the muon (electron). In analogy with a two-body mass, the W boson transverse mass is defined using only the transverse momentum vectors as mT=√2 ( pℓT pνT−→p ℓT⋅→p νT) (53). High-purity samples of W bosons are obtained with the requirements 30<pℓT<55 GeV, 30<pνT<55 GeV, |→u|<15 GeV, and 60 < mT < 100 GeV. This selection retains samples containing precise MW information and low backgrounds. The final samples of W and Z bosons consist of 1,811,700 (66,180) W→eν (Z→ee) candidates and 2,424,486 (238,534) W→μν (Z→μμ) candidates.
Simulation of physical processes
The data distributions of mT, pℓT, and pνT are compared with corresponding simulated line shapes (“templates”) as functions of MW from a custom Monte Carlo simulation that has been designed and written for this analysis. A binned likelihood is maximized to obtain the mass and its statistical uncertainty. The kinematic properties of W and Z boson production and decay are simulated using the resbos program (54–56), which calculates the differential cross section with respect to boson mass, transverse momentum, and rapidity for boson production and decay. The calculation is performed at next-to-leading order in perturbative quantum chromodynamics (QCD), along with next-to-next-to-leading logarithm resummation of higher-order radiative quantum amplitudes. resbos offers one of the most accurate theoretical calculations available for these processes. The nonperturbative model parameters in resbos and the QCD interaction coupling strength αs are external inputs needed to complete the description of the boson pT spectrum and are constrained from the high-resolution dilepton pℓℓT spectrum of the Z boson data and the pWT data spectrum. EM radiation from the leptons is modeled with the photos program (57), which is calibrated to the more accurate horace program (58, 59). We use the nnpdf3.1 (60) parton distribution functions (PDFs) of the (anti)proton, as they incorporate the most complete relevant datasets of the available next-to-next-to-leading order (NNLO) PDFs. Using 25 symmetric eigenvectors of the nnpdf3.1 set, we estimate a PDF uncertainty of 3.9 MeV. We find that the ct18 (61), mmht2014 (62), and nnpdf3.1 NNLO PDF sets produce consistent results for the W boson mass, within ±2.1 MeV of the midpoint of the interval spanning the range of values. The model-dependent nature of the analysis implies that future improvements or corrections in any relevant theoretical modeling can be used to update our measurement quantifiably [see section IV of (63)].
The custom simulation includes a detailed calculation of the lepton and photon interactions in the detector (39, 43, 64), as well as models describing their individual position measurements within the COT. The COT position resolution as a function of radius is determined using muon tracks from Υ meson, W boson, and Z boson decays. All wire positions in the COT are measured with 1-μm precision using an in situ sample of cosmic ray muons (65), in addition to the electron tracks from W boson decays. The difference between electron and positron track momenta relative to their measured energy in the calorimeter (which is independent of charge) strongly constrains certain modes of internal misalignment in the COT.
Momentum and energy calibration
The track momentum measurement in the COT is calibrated by measuring the masses of the J/ψ and Υ(1S) mesons reconstructed in their dimuon decays and comparing them with the known values (10). These meson mass measurements are performed with maximum-likelihood fits to the dimuon mass distributions from data, using templates obtained from the custom simulation. Measurements of these masses as functions of muon momenta are used to correct for small inaccuracies in the magnetic field map, the COT position measurements, and the modeling of the energy loss by particles traversing the detector. A mismodeling of the energy loss would lead to a bias linear in the mean inverse pT of the two muons. No such bias is observed after applying the magnetic field nonuniformity, COT, and energy-loss corrections (Fig. 2A). The curvature q/pT measured by the COT, where q is the particle charge, is an analytic function of the true curvature. The curvature response function analytically yields a linear dependence of the measured invariant mass on p−1T, and higher-order terms in p−1T are negligible. The correction for the fractional deviation of the measured momentum from its correct value, Δp/p≡pmeasured/ptrue−1, is inferred from the comparison of the measured meson masses to their more-precise world-average masses. The Δp/p corrections extracted from the individual J/ψ and Υ(1S) invariant mass fits are consistent with each other, and the results are combined to obtain Δp/p=(−1393±26) parts per million (ppm).

Fig. 2. Calibration of track momentum and electron’s calorimeter energy.
(A) Fractional deviation of momentum Δp/p (per mille) extracted from fits to the J/ψ→μμ resonance peak as a function of the mean muon unsigned curvature 〈1/pμT〉 (blue circles). A linear fit to the points, shown in black, has a slope consistent with zero (17 ± 34 keV). The corresponding values of Δp/p extracted from fits to the ϒ→μμ and Z→μμ resonance peaks are also shown. The combination of all of these Δp/p measurements yields the momentum correction labeled “combined,” which is applied to the lepton tracks in W boson data. Error bars indicate the uncorrelated uncertainties (total uncertainty) for the individual boson measurements (combined correction). (B) Distribution of E/p for the W→eν data (points) and the best-fit simulation (histogram) including the small background from hadrons misreconstructed as electrons. The arrows indicate the fitting range used for the electron energy calibration. The relative energy correction ΔSE, averaged over the calibrated W and Z boson data [see fig. S13 in (63)], is compatible with zero. In this and other figures, PKS refers to the Kolmogorov-Smirnov probability of agreement between the shapes of the data and simulated distributions.
The combined momentum calibration is used to measure the Z boson mass in the dimuon channel (Fig. 3A), which is blinded with a random offset in the range of −50 to 50 MeV until all analysis procedures are established. The unblinded measurement is MZ=91,192.0±6.4stat±4.0syst MeV (stat, statistical uncertainty; syst, systematic uncertainty), which is consistent with the world average of 91,187.6±2.1 MeV (10, 44) and therefore provides a precise consistency check. Systematic uncertainties on MZ result from uncertainties on the longitudinal coordinate measurements in the COT (1.0 MeV), the momentum calibration (2.3 MeV), and the QED radiative corrections (3.1 MeV). The latter two sources are correlated with the MW measurement. The Z→μμ mass measurement is then included in the final momentum calibration. The systematic uncertainties stemming from the magnetic field nonuniformity dominate the total uncertainty of 25 ppm in the combined momentum calibration.

Fig. 3. Decay of the Z boson.
(A and B) Distribution of (A) dimuon and (B) dielectron mass for candidate Z→μμ and Z→ee decays, respectively. The data (points) are overlaid with the best-fit simulation template including the photon-mediated contribution (histogram). The arrows indicate the fitting range.
After track momentum (p) calibration, the electron’s calorimeter energy (E) is calibrated using the peak of the E/p distribution in W→eν (Fig. 2B) and Z→ee [fig. S13 in (63)] data. Fits to this peak in bins of electron ET determine the electron energy calibration and its dependence on ET. The radiative region of the E/p distribution (E/p > 1.12) is fitted to measure a small correction (≈5%) to the amount of radiative material traversed in the tracking volume. The EM calorimeter resolution is measured using the widths of the E/p peak in the W→eν sample and of the mass peak of the Z→ee sample.
We use the calibrated electron energies to measure the Z boson mass in the dielectron channel (Fig. 3B), which is also blinded with the same offset as used for the dimuon channel. The unblinded result, MZ=91,194.3±13.8stat±7.6syst MeV, is consistent with the world average, providing a stringent consistency check of the electron energy calibration. Systematic uncertainties on MZ are caused by uncertainties on the calorimeter energy (6.5 MeV) and track momentum (2.3 MeV), on the z coordinate measured in the COT (0.8 MeV), and on QED radiative corrections (3.1 MeV). Measurements of the Z boson mass using the dielectron track momenta, and comparisons of mass measurements using radiative and nonradiative electrons, provide consistent results. The final calibration of the electron energy is obtained by combining the E/p-based calibration with the Z(→ee) mass-based calibration, taking into account the correlated uncertainty on the radiative corrections.
The spectator partons in the proton and antiproton, as well as the additional (≈3) pˉp interactions in the same collider bunch crossing, contribute visible energy that degrades the resolution of →u. These contributions are measured from events triggered on inelastic pˉp interactions and random bunch crossings, reproducing the collision environment of the W and Z boson data. Because there are no high-pT neutrinos in the Z boson data, the →pT imbalance between the →pℓℓT and →u in Z→ℓℓ events is used to measure the calorimeter response to, and resolution of, the initial-state QCD radiation accompanying boson production. The simulation of the recoil vector →u also requires knowledge of the distribution of the energy flow into the calorimeter towers impacted by the leptons, because these towers are excluded from the computation of →u. This energy flow is measured from the W boson data using the event-averaged response of towers separated in azimuth from the lepton direction.
Extracting the W boson mass
Kinematic distributions of background events passing the event selection are included in the template fits with their estimated normalizations. The W boson samples contain a small contamination of background events arising from QCD jet production with a hadron misidentified as a lepton, Z→ℓℓ decays with only one reconstructed lepton, W→τν→ℓνˉνν, pion and kaon decays in flight to muons (DIF), and cosmic-ray muons (τ, tau lepton; ˉν, antineutrino). The jet, DIF, and cosmic-ray backgrounds are estimated from control samples of data, whereas the Z→ℓℓ and W→τν backgrounds are estimated from simulation. Background fractions for the muon (electron) datasets are evaluated to be 7.37% (0.14%) from Z→ℓℓ decays, 0.88% (0.94%) from W→τν decays, 0.01% (0.34%) from jets, 0.20% from DIF, and 0.01% from cosmic rays.
The fit results (Fig. 4) are summarized in Table 1. The MW fit values are blinded during analysis with an unknown additive offset in the range of −50 to 50 MeV, in the same manner as, but independent of, the value used for blinding the Z boson mass fits. As the fits to the different kinematic variables have different sensitivities to systematic uncertainties, their consistency confirms that the sources of systematic uncertainties are well understood. Systematic uncertainties, propagated by varying the simulation parameters within their uncertainties and repeating the fits to these simulated data, are shown in Table 1. The correlated uncertainty in the mT (pℓT, pνT) fit between the muon and electron channels is 5.8 (7.9, 7.4) MeV. The mass fits are stable with respect to variations of the fitting ranges.

Fig. 4. Decay of the W boson.
(A to C) Distributions for mT (A), pℓT (B), and pνT (C) for the muon channel. (D to F) Same as in (A) to (C) but for the electron channel. The data (points) and the best-fit simulation template (histogram) including backgrounds (shaded regions) are shown. The arrows indicate the fitting range.
DistributionW boson mass (MeV)χ2/dofmT(e,ν)80,429.1±10.3stat±8.5syst39/48pℓT(e)80,411.4±10.7stat±11.8syst83/62pνT(e)80,426.3±14.5stat±11.7syst69/62mT(μ,ν)80,446.1±9.2stat±7.3syst50/48pℓT(μ)80,428.2±9.6stat±10.3syst82/62pνT(μ)80,428.9±13.1stat±10.9syst63/62Combination80,433.5±6.4stat±6.9syst7.4/5Expand for more
Table 1. Individual fit results and uncertainties for the MW measurements.
The fit ranges are 65 to 90 GeV for the mT fit and 32 to 48 GeV for the pℓT and pνT fits. The χ2 of the fit is computed from the expected statistical uncertainties on the data points. The bottom row shows the combination of the six fit results by means of the best linear unbiased estimator (66).
Simulated experiments are used to evaluate the statistical correlations between fits, which are found to be 69% (68%) between mT and pℓT (pνT) fit results and 28% between pℓT and pνT fit results (43). The six individual MW results are combined (including correlations) by means of the best linear unbiased estimator (66) to obtain MW=80,433.5±9.4 MeV, with χ2/dof = 7.4/5 corresponding to a probability of 20%. The mT, pℓT, and pνT fits in the electron (muon) channel contribute weights of 30.0% (34.2%), 6.7% (18.7%), and 0.9% (9.5%), respectively. The combined result is shown in Fig. 1, and its associated systematic uncertainties are shown in Table 2.
SourceUncertainty (MeV)Lepton energy scale3.0Lepton energy resolution1.2Recoil energy scale1.2Recoil energy resolution1.8Lepton efficiency0.4Lepton removal1.2Backgrounds3.3pZT model1.8pWT/pZT model1.3Parton distributions3.9QED radiation2.7W boson statistics6.4Total9.4Expand for more
Table 2. Uncertainties on the combined MW result.
OPEN IN VIEWERDiscussion
The dataset used in this analysis is about four times as large as the one used in the previous analysis (41, 43). Although the resolution of the hadronic recoil is somewhat degraded in the new data because of the higher instantaneous luminosity, the statistical precision of the measurement from the larger sample is still improved by almost a factor of 2. To achieve a commensurate reduction in systematic uncertainties, a number of analysis improvements have been incorporated, as described in table S1. These improvements are based on using cosmic-ray and collider data in ways not employed previously to improve (i) the COT alignment and drift model and the uniformity of the EM calorimeter response, and (ii) the accuracy and robustness of the detector response and resolution model in the simulation. Additionally, theoretical inputs to the analysis have been updated. Upon incorporating the improved understanding of PDFs and track reconstruction, our previous measurement is increased by 13.5 MeV to 80,400.5 MeV; the consistency of the latter with the new measurement is at the percent probability level.
In conclusion, we report a new measurement of the W boson mass with the complete dataset collected by the CDF II detector at the Fermilab Tevatron, corresponding to 8.8 fb−1 of integrated luminosity. This measurement, MW=80,433.5±9.4 MeV, is more precise than all previous measurements of MW combined and subsumes all previous CDF measurements from 1.96-TeV data (38, 39, 41, 43). A comparison with the SM expectation of MW=80,357±6 MeV (10), treating the quoted uncertainties as independent, yields a difference with a significance of 7.0σ and suggests the possibility of improvements to the SM calculation or of extensions to the SM. This comparison, along with past measurements, is shown in Fig. 5. Using the method described in (45), we obtain a combined Tevatron (CDF and D0) result of MW=80,427.4±8.9 MeV. Assuming no correlation between the Tevatron and LEP measurements, their average becomes MW=80,424.2±8.7 MeV.
Acknowledgments
We thank A. Accardi, C. Carloni Calame, S. Carrazza, G. Ferrera, S. Forte, Y. Fu, L. Harland-Lang, J. Isaacson, P. Nadolsky, J. Rojo, N. Sato, S. Sen, R. Thorne, A. Vicini, Z. Was, G. Watt, and C.-P. Yuan for helpful discussions. This document was prepared by the CDF Collaboration using the resources of the Fermi National Accelerator Laboratory (Fermilab), a US Department of Energy, Office of Science, HEP User Facility. Fermilab is managed by Fermi Research Alliance, LLC (FRA), acting under contract no. DE-AC02-07CH11359. We thank the Fermilab staff and the technical staffs of the participating institutions for their vital contributions.
Funding: This work was supported by the US Department of Energy and National Science Foundation; the Italian Istituto Nazionale di Fisica Nucleare; the Ministry of Education, Culture, Sports, Science and Technology of Japan; the Natural Sciences and Engineering Research Council of Canada; the National Science Council of the Republic of China; the Swiss National Science Foundation; the Alfred P. Sloan Foundation; the Bundesministerium für Bildung und Forschung, Germany; the National Research Foundation of Korea; the Science and Technology Facilities Council and the Royal Society, UK; the Russian Foundation for Basic Research; the Ministerio de Ciencia e Innovación, and Programa Consolider-Ingenio 2010, Spain; the Slovak R&D Agency; the Academy of Finland; and the Australian Research Council (ARC).
Author contributions: All authors contributed to various aspects of the experiment’s construction and operation, data acquisition and reconstruction, review of the analysis, and approval of the manuscript. A.V.K. led the analysis and wrote the paper.
Competing interests: All authors declare that they have no competing interests.
Data and materials availability: No materials are involved in the results presented. Data and code have been deposited in the Zenodo repository (67) and are based on the functionality of the CERN ROOT analysis package version 5.34/12.
License information: This work is licensed under a Creative Commons Attribution 4.0 International (CC BY 4.0) license, which permits unrestricted use, distribution, and reproduction in any medium, provided the original work is properly cited. To view a copy of this license, visit https://creativecommons.org/licenses/by/4.0/. This license does not apply to figures/photos/artwork or other content included in the article that is credited to a third party; obtain authorization from the rights holder before using such material.
Recommend
About Joyk
Aggregate valuable and interesting links.
Joyk means Joy of geeK